Woe is us.
Our utility bills are up. Politicians want to drill for oil in the Gulf of Mexico and other ecologically sensitive areas. Global warming has already begun to harm the planet. As we make ever greater demands on our limited resources, most of us worry about the long-range consequences. What can be done?
Scientists have been exploring many options, from more practical applications of solar energy to safer nuclear power plants. Also laboring toward a solution, at the MagLab and elsewhere, are physicists using mammoth magnets and a host of other tools. With them they hope to harness the power of a phenomenon they have experimented with for nearly a century and theorized about for even longer, but have yet to master, or even fully understand: superconductivity. Think of it as electricity on steroids.
No, wait – that’s not quite right. While the word “steroids” does convey the incredible power of superconductivity, it also, incorrectly, suggests substantial sweat and effort. And the beauty of this physics phenomenon is in the lack of effort required for superconducting current to flow.
Here’s another stab at defining superconductivity. It’s like those perfect days that sometimes come our way, when everything goes magically right, when all obstacles are removed from our paths and we fly through the hours, accomplishing every task almost effortlessly, as if on autopilot.
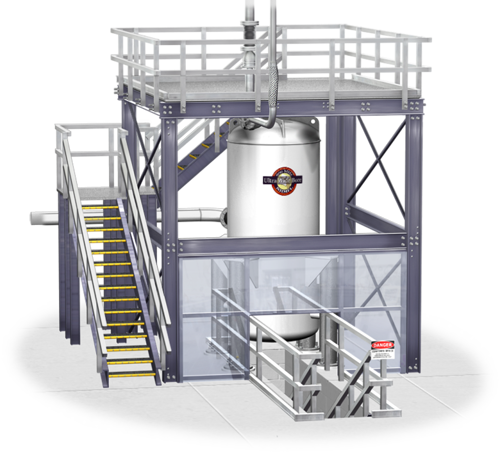
The 900 MHz ultrawide bore magnet
Yes, that is superconductivity: great efficiency achieved with great ease. In fact, when you have a superconducting current going, it can keep on going and going and going – long after you unplug the machine, long after the Energizer Bunny calls it quits, years after, bored senseless, you stop waiting around to see how long it would last.
Sounds miraculous, doesn’t it? Well, it's a reality. In fact, the MagLab has superconductivity to thank for one of its most powerful tools: Its 900 MHz ultra-wide bore magnet. One of the most powerful superconducting magnets in the world, it is used for important research in nuclear magnetic resonance (NMR). Since it was first charged in July 2004, it has been continually conducting electricity by itself – no plug needed.
Here’s the rub: While superconductivity, once achieved, runs like a dream, creating the environment and tools that make it possible is a substantial chore.
Physics Factoid
In 2003, the world consumed 14,768 billion kilowatt hours of electricity. Assuming high economic growth, that number is projected to rise to 28,870 billion by the year 2025. (If you use a 100-watt light bulb for one hour, you have used 100 watts of power, or 0.1 kilowatt hours.)
The pursuit of superconductivity makes for an exciting story, full of suspense, red herrings, scandalous behavior on the part of certain atomic particles, a take-home message on the benefits of cooperation and, potentially, a very happy ending. And, as do all good stories, ours has a hero. We will call him, simply, SuperConductor.
But we are getting ahead of ourselves a bit here. Before we can comprehend our caped crusader’s prodigious powers, we need to meet his mild-mannered cover: Spark Kent. Spark’s day job is at the local power plant, conducting electricity to our community’s homes and businesses in pretty much the same ho-hum way it has been done since the late 1800s. Though Spark works hard, it’s not hard enough … not with the energy problems facing the planet. The atoms are restless, the electrons are unproductive: Someone needs to come to the rescue.
For those of you needing a quick refresher course in the behavior of atoms – knowledge essential to understanding superconductivity – proceed to the next section. Otherwise, feel free to use the drop-down menu at the top of the page to jump ahead.
Electron Done Me Wrong: Atoms in a Nutshell
Understanding electricity means first recalling how atoms work. Though you could never tell by looking at them (at least with the naked eye), these atoms are as active as the protagonists of “Desperate Housewives.”
At the core of this basic building block of matter is, of course, a nucleus, comprised (in part) of one or more positively-charged protons. Around these orbit electrons – the negative answer to the proton’s positive disposition. Depending on how many electrons an atom has, it may have one orbit of electrons or several. Hydrogen has a single electron in its one little orbit, while uranium has 92 electrons across seven orbits.

Aluminum Atom
What matters for our purposes, though, is not how big the atom is, but how many electrons are in its outermost (or valence) orbit, in relation to how many it could have there. Nature is pretty specific about the maximum number of electrons it will accommodate in a given orbit. Two is tops for the first orbit, and eight is tops for the second. The third orbital would be full at eight, too (though it could squeeze in twice that many for parties). Let's consider an aluminum atom, for example. It has 13 electrons: two (the maximum) in the first orbit, eight (the maximum) in the second orbit, and the remainder – three – in its third and final orbit, which isn't full (it could fit at least five more).
Generally, the number of protons in an atom equals the number of electrons, and their charges cancel each other out. But such stability is not always the case – what kind of story would we have if it was? If you think of an atom as a family (The Atom Family, if you will) made up of particles, the protons pretty much stay at home. Electrons, however, sometimes behave like the can’t-help-myself bad boys in a maudlin country song: always hooking up with other opposites, or just hitting the road to roam free. In fact, they’re often called free electrons.
It’s a story older than – ahem – Atom and Eve: In the right situation, an electron parts company with its home atom, leaving it with bad memories and a net positive charge. Atoms that have such a charge are called ions. We’ve just described a cation (a positively-charged ion), but there are also anions (negatively charged ions, with more electrons than protons).
Physics Factoid
Electrons are almost inconceivably tiny and light. A proton is gigantic in comparison, with a mass about 1,836 times that of an electron. It would take many, many, many billions of electrons to equal a single gram.
Some inherently stable materials (wood is an example) are comprised of atoms whose electrons are what you might call the marrying type – they tend to stay put in their shells. These materials are insulators. Due to the particular arrangement of electrons orbiting their nuclei – the outermost orbit is happily full – they are not inclined to accommodate lone electrons cruising the neighborhood. Other materials, however, are more libertine. In them, electrons have the potential to move about easily from one atom to another, or to hit the electron highway. These are conductors. That’s the case with our aluminum atom (and metals in general). One or more of its three free electrons might decide to wander off; likewise, the valence shell has room to accommodate visitors.
All of which brings us back to electricity.
Fuel of Hard Knocks: Electricity in a Nutshell
Let’s take a closer look at our aluminum atom.
Some of the hopping-about activity we just described takes place in a length of aluminum wire sitting on a table. You don’t need to plug it in – the energy inherent in the average room is enough to excite the electrons. The valence electrons in the aluminum migrate back and forth from one atom to the next.
Now, you can kick things up a notch by introducing a battery into the situation. If you connect one end of your wire to the positive end, and the other to the negative end, those free electrons in your aluminum will begin moving, all in the same direction. The battery has an excess of electrons on the negative terminal and lack of them on the positive, so the negative side pushes the negatively-charged particles into the aluminum and the positive side pulls them out. The aluminum conductor provides the highway for the electrons to travel on. This creates a circuit of streaming electrons. Voilà: electricity to fuel your radio or flashlight.

Free electrons moving among aluminum atoms
In truth, “streaming” is not the best word to describe what the electrons are doing. The verb used by scientists is drift – and for good reason. In the current lighting up a household light bulb, the electrons are taking their sweet time, moseying along at only a few inches an hour! (The fact that they can power your mixer is due not so much to their speed as to the vast quantity of electrons that are moving.)
Why such slowpokes? The problem is that it’s a veritable demolition derby out there, with electrons bumping into atoms (or other free electrons) in their attempt to answer the siren call of the battery’s positive charge. That’s what’s called resistance. The atoms are all the more difficult to avoid because they won’t sit still: Electrons still in their orbit are busy circling their nuclei, and the nuclei themselves vibrate somewhat. As a result, the electrons get knocked off course frequently. The energy they give off in the process is not electricity, but heat – a big problem for our Spark Kent. That’s why the electric wires in your house would be slightly warm to the touch if they weren’t wrapped in insulating plastic. So heat is a byproduct of electricity that is sometimes desirable (in electric blankets or stoves) and sometimes not (in computers and other electronics). To see this concept illustrated, check out this interactive tutorial on resistance.
Physics Factoid
Free electrons in a current travel along the surface of a wire, because they repel each other as much as possible as they move toward the positive charge. This is called the “skin effect.”
The collisions contribute to another important inefficiency to these currents. Electrons tend to take the scenic route. Imagine a guy from Tallahassee who wants to go see his new girlfriend in Los Angeles. His car is in the shop and he’s reduced to hitchhiking (he’s a desperate man), so instead of shooting straight down I-10, he must settle for whatever ride he can get. He ends up zigzagging up to Birmingham, then down to Biloxi, then back up to Little Rock, then backwards to Memphis, then down to – well, you get the picture.
The bottom line of this electron behavior is about a 7 percent loss of potential current. As you might imagine, billions of dollars could be saved every year in the United States alone if not for that loss. Wouldn’t it be super if someone could find a way around that?
It’s a Bird, It’s a Plane, It’s … : Superconductivity in a Nutshell
Someone has! Enter our hero, SuperConductivity, to save the day! His power: plowing past obstacles as if they weren’t even there.
The key to achieving superconductivity is keeping fidgety atoms out of the way of the flow of electrons. The hotter those atoms get, the more fidgety they get: That’s why the atoms in your ice cubes are slow-pokes compared to those in your tea, which in turn trudge like turtles compared to the atoms zipping about in the steam coming out of the kettle.

Temperature Scales
So to get atoms to truly chill out, you need to turn the A/C up.
Way up.
The fundamental laws of physics tell us that you can never suck all the energy out of a chunk of matter. But you can come pretty close and, as it turns out, pretty close is close enough.
How cold is close enough?
First, let’s define the bottom of the temperature barrel. As far as we know, there is no limit to how hot the universe could get. But, thanks to a gentleman named William Thomson (Lord Kelvin to you), we know that there is a definite limit to how cold it can get. Scientists call it 0 kelvin (0 K), or absolute zero. You might call it -273 degrees Celsius, -460 degrees Fahrenheit, or just gosh darn frosty. By comparison, the coldest temperature ever recorded on Earth was -129 degrees Fahrenheit.
You may be relieved to learn that you don’t need to get all the way down to absolute zero in order to achieve superconductivity. This fact came as quite a surprise, though, to the scientist who first, quite unexpectedly, achieved that state, which until then had only been theoretical. That happened in 1911.
Dutch physicist Heike Kamerlingh Onnes was a pioneer in liquefying hard-to-liquefy gasses. At the time he was the first, and only, person to create and work with liquid helium, which requires a teeth-chattering 4 K. He wanted to see how metals (for this experiment he used mercury) behave when exposed to such extremes.

As you’ve guessed by now, Onnes discovered that the metal lost all resistance, and electrons flowed through freely. As a result, he became known as the father of superconductivity.
What can speed through matter in a single bound? Not a bird, not conventional electric current, but our hero, SuperConductivity!
Physics Factoid
Not all substances have the superconductive property. Among the elements in the periodic table, 57 are known (as of May 2009) to be capable of superconductivity, but some superconduct only under high pressure or in modified forms, such as thin films or nanotubes, as shown in the table below.
As a hero, SuperConductivity shows tremendous potential. A lot of famous and not-so-famous scientists have for a century worked to unlock its secrets. They have made considerable progress, creating experimental currents that run for years, developing highly valuable applications such as magnetic resonance imaging (MRI) and “levitating” maglev trains. The age of superconductivity is still young, but it is here.
But like other superheroes, SuperConductivity has his nemesis. Let’s call him Iceman.
It’s true that extreme cold is necessary for superconductivity. But Iceman is still our bad guy because he makes the process quite difficult (liquid helium is expensive and cumbersome to work with) and prohibitively impractical. Scientists have been battling this enemy ever since 1911, as we’ll see in a bit.
Still, Onnes’ news was revolutionary, holding all kinds of promise for humankind. It would be many decades, though, before scientists could begin to develop applications for the discovery. Heck – for many years they didn’t even know how superconductivity really worked.
Two by Two: Cooper Pairs
That changed in 1957, when a trio of researchers, pursuing a hunch that electrons in a superconductive state were actually attracting each other, hit on a pretty convincing hypothesis. For this they got a theory named after them (Bardeen-Cooper-Schrieffer, for John Bardeen, Leon Cooper and Robert Schrieffer) and, as a little added bonus, a Nobel Prize.
Physics Factoid
Physicists tend not to think about how cold things are – even when they’re near absolute zero – but how hot they are; cold is nothing but the absence of heat. The coldest our universe gets naturally, scientists believe, is about 3 kelvin. However, they have created temperatures within a tiny fraction of a degree above absolute zero in laboratories, including at the MagLab's Millikelvin Facility and our High B/T Facility.
The idea behind BCS, of course, flies in the face of everything you have learned about particle physics. Opposites attract, like particles repel. That’s what makes atoms spin ’round.
Yet to every rule there’s an exception, or two or three (welcome to physics!). Chalk this one up to the extreme temperatures in which superconductivity is achieved.
Put on your parka and mittens and we’ll go take a look.
The atoms of metals, in their solid state, have a crystalline lattice structure. Picture a tower of interconnected cubes made with Tinker Toys, the little wooden discs being the atoms. The space between the discs represents the potential paths for our electrons. Because this metal is surrounded by liquid helium, the atoms are barely moving.
There wouldn’t be anything "super" about superconductivity if the electrons moseyed through this lattice one at a time. What actually happens, according to BCS theory, is a kind of buddy system: The electrons pair up, zipping through the lattice with greater efficiency, forming a very fluid stream. Scientists call these electrons Cooper pairs. To reprise our earlier metaphor, think how much easier it is to strike up a conversation with someone new if, rather than going solo, you have a friend providing support.
Watch the video below for a depiction of how Cooper pairs form.
Generally speaking, this is what it looks like: When an electron enters the lattice and passes between two positively-charged atoms, those cations get tugged just slightly toward the electron, and each other. The ions do not capture the electron with their positive charms; nor do they impede it. Rather, at superconducting temperatures, they nudge it along by creating a positively-charged wave, called a phonon, behind the electron as it passes. This positively-charged phonon, in turn, draws a second electron into the picture, which piggy-backs on the first electron’s momentum. Thus they become buddy-buddy, and continue traveling that way.
You might picture it this way: Our first electron tries to start a conversation with the positively-charged ions. The ions lean in a bit to hear what he has to say, decide they’re not interested, and go back to where they were, happy to let the boorish electron (and its buddy) move on and try their luck elsewhere. The phenomenon of Cooper pairs, by its very quantum nature, is hard to visualize, but the below animation communicates the main ideas about what happens in this state.
The electrons are not attracted to each other: The phonon acts as the bond. This bond is pretty stable, and certainly more efficient than single electrons zigzagging their way one by one through the crowd of cations. The electrons, you might say, watch out for each other, neither one letting his buddy stray from the straight and narrow.
There is another important aspect of this theory: These pairs don’t cooperate only with each other, but also with other Cooper pairs. Simply speaking, the pairs can overlap, or even include other pairs within a longer pairing. This creates one cohesive group of paired electrons flowing with exquisite efficiency through the superconductor – as a single wave. The transformation is such that materials in superconducting mode are considered to be in a phase of matter all their own, distinct from solids, gasses or liquids.
The Iceman Succumbeth: High-Temperature Superconductivity
Even before BCS theory came along, scientists began trying to create superconductivity at higher temperatures. They knew that if mercury became superconductive at 4 K, other metals or alloys might, under the right conditions, become superconductive at more reasonable temperatures. Their great hope was to create superconductivity at a temperature of 77 K. While still frigid by most standards, that temperature could be created by liquid nitrogen. And liquid nitrogen is considerably cheaper and easier to work with than liquid helium.
Physics Factoid
Liquid nitrogen is much more convenient and cheaper than liquid helium because nitrogen is all around us. In fact, although we’re used to equating air with oxygen, nitrogen makes up more than two-thirds of the stuff we breathe into our lungs. Helium, by contrast, is much harder to come by, and must be extracted from gases mined in the Great Plains and elsewhere.
Over the course of the last century, scientists chipped away at the evil Iceman. They made important gains, but it was slow-going. And in the grand scheme of things, they were baby steps. Iceman remained a formidable opponent.
It wasn’t until 1986 that a giant, Armstrongian step was taken in the field.
That year, a pair of IBM scientists published research showing they had achieved superconductivity at about 30 K. More surprising than the temperature reached, though, was the material they reached it with: a ceramic oxide.
Until then, few scientists believed that using ceramics (inorganic non-metals) for superconductivity held much promise. But when others confirmed the IBM results, those doubters changed their minds pretty fast. A mad rush ensued during which researchers across the world renewed their efforts to reach that Holy Grail: superconductivity at 77 K.
Within a year, it happened, and a new field of research was born: high-temperature superconductivity.
In the years since then, scientists have been busy. They continue to push the envelope on superconductivity: As of 2006, the record of 138 K, achieved in 1994, was still standing. (Scientists have actually achieved superconductivity at temperatures as warm as 164 K, but only under high pressure).

But breaking through the high-temperature barrier represents, for our hero, SuperConductivity, the end of a battle with Iceman, not the whole war. The next great achievement – the holier-than-thou grail, if you will – is superconductivity at room temperature, without liquid nitrogen. That is many a scientist’s dream.
Another feat scientists fantasize about is the discovery of how high-temperature superconductivity works. Experts agree that BCS theory only explains superconductivity up to 40 K. A number of researchers have proffered theories to account for superconductivity higher up the Kelvin scale, but none have succeeded in settling the matter within the scientific community.
Nothing Up My Sleeve: The Meissner Effect
Loss of resistance is a pretty cool phenomenon. But that’s not all superconductivity has in its bag of tricks. Wait till you get a load of the Meissner Effect.

Indeed, demonstrations of this effect look like a page torn from a magician's notebook. Wacky scientists have made all manner of things levitate using the Meissner Effect, including sumo wrestlers!
The magic is known as diamagnetism, when a superconducting material excludes the magnetic force of another magnet, thereby forcing that magnet to float on air. You might say that the superconductor adopts a "This town ain't big enough for both of us" mentality.
If you think levitating a small magnet is an impressive feat, try a train! That's exactly what numerous groups of engineers and scientists across the globe have been doing for years, exploiting this property for use in high-speed maglev trains that levitate above the track. By using magnets – either superconducting magnets or regular electromagnets requiring electricity – they eliminate the friction that slows down all other transportation by land.
Split Personality: Type 1 and Type 2 Superconductors
Now that you’ve learned about low-temperature and high-temperature superconductivity and the Meissner Effect, you’re ready for us to throw another concept at you: Type 1 and Type 2 superconductors.
All high-temperature superconductors fall into the Type 2 category, as do all superconducting alloys; superconductors made of just one element (mercury and aluminum, for example) are Type 1’s. But these two classifications shouldn’t get confused. Let’s back up a little.
You know already that temperature can determine whether or not a material becomes superconductive. Well, the presence of a magnetic field can also make or break a material’s superconductivity.
Physics Factoid
This phenomenon is referred to more formally as the Meissner-Ochsenfeld Effect, after the two Germans who discovered it in 1933, Walther Meissner and Robert Ochsenfeld.
You know from the Meissner Effect that a superconductor can expel an outside magnetic force. What we didn’t tell you is that, if that magnetic force gets high enough, the tables turn: The magnetic field gets the better of the superconductor, which then loses its superconductivity and begins conducting electricity normally again.
The strength of the magnetic field required to break the material’s superconductivity is called the critical field.
What separates Type 1 and Type 2 superconductors is the way in which they revert to normal conductivity in the presence of a critical field.
The way Type 1 superconductors operate is pretty cut and dried (as cut and dried as physics can get!). Let’s take our friend mercury. As you know, this element is superconducting at 4.2 K. But expose it to a magnetic field of .041 tesla or higher, and all bets are off; those Cooper pairs break up and the mercury immediately reverts to normal conduction.
Type 2 superconductors are a little more complicated. They have not one, but two critical fields. Let’s take as an example a material widely used in the superconducting magnets of MRI machines: niobium-tin, or Nb3Sn. This alloy becomes superconducting at 18 K (assuming the absence of any significant magnetic field).
Below Nb3Sn’s lower critical field of 0.01 tesla, the alloy remains fully superconducting. Above its upper critical field of about 29 tesla, it ceases superconducting entirely. But in between these two fields the material enters what’s known as a mixed state – essentially, part of it conducts electricity normally, part of it is superconducting.
Physics Factoid
Find the terms “Type 1” and “Type 2” too …. cold? Here’s an alternative: The former is sometimes referred to as “soft,” the latter as “hard.”
This mixed state comes about because the superconducting material no longer fully excludes the outside magnetic field; as the field strength increases and approaches the upper critical field, the more the field penetrates the superconductor. The closer to the upper critical field you get, the less superconducting the material becomes.
So unlike Type 1 superconductors, which have a take-it-or-leave-it approach to superconductivity, Type 2 superconductors are willing to spend more time in a middle ground.
The long and the short of this, though, is that Type 2 superconductors generally can sustain superconductivity in the presence of much higher magnetic fields. This is of tremendous consequence to Magnet Lab scientists and others who need high magnetic fields for their experiments. All of the superconducting magnets used in high-field research are Type 2.
Superconductivity is a unique and powerful tool in scientific research, and promises great benefits for the planet. As the force that makes MRI possible, superconductivity has already improved – and often saved – the lives of countless patients and their doctors. Other applications of the technology have been under development for years, including maglev “levitating” trains and superconducting power cables that could revolutionize electricity across the planet. You may well reap the benefits of this research in your lifetime. Click on any of these links, or check out some of the resources listed below, to explore other aspects of superconductivity.
Thanks to this story's science adviser: Dr. Scott Hannahs, Research Associate and Chief of User Research Instrumentation at the Magnet Lab.
By Kristen Coyne